Methan als Feststoff
Kohlenstoff ist grau dargestellt, Wasserstoff weiß.
Formel: CH4
Es wird auch die Elementarzelle dargestellt, das ist der kleinstmögliche Block von Atomen,
der dann gestapelt den Kristall ergibt. Sie hat in diesem Fall die Form eines Würfels.
Dargestellt ist die Modifikation Methan I. Dies ist die Modifikation, die entsteht, wenn man Methan bei normalem Athmosphärendruck
abkühlt. (Man beachte, dass die Druckskala im beigefügten Phasendiagramm bei 0,01 GPa beginnt, das entspricht dem hundertfachen
Athmosphärendruck!).
Phasendiagramm für Methan. Nach: R. Bini & G. Pratesi (1997): High-pressure infrared study of solid methane: Phase diagram up
to 30 GPa. Physical Review B, 55 (22), 14800-14809.
In dieser Modifikation können sich die Methanmoleküle frei drehen; die Positionen der Wasserstoffatome sind also im
Kristallgitter nicht festgelegt. In der Darstellung wurde dies so berücksichtigt, dass sich die einzelnen Methanmoleküle jeweils
in einer unterschiedlichen, zufällig gewählten Position befinden. Aufgrund dieser Beweglichkeit sind die Kristalle dieser
Modifikation plastisch. Bei weiterer Abkühlung verlieren die Moleküle nach und nach ihre freie Drehbarkeit, wodurch sich die
dann folgenden Modifikationen ergeben.
Betrachten Sie die Kristallstruktur auch bei der Darstellung mit 100% Raumfüllung der Atome! Vergleichen Sie außerdem
mit den anderen Alkanen.
Die Darstellung beruht auf eigenen Berechnungen nach den kristallographischen Daten in: A. I. Kitajgorodskij (1955): Organicheskaja
Kristallokhimija. Izdatel'stvo Akademii Nauk SSSR, zitiert nach: Crystallography Open Database (COD), http://www.crystallography.net/
Ethan als Feststoff
Kohlenstoff ist grau dargestellt, Wasserstoff weiß.
Formel: C2H6
Es wird auch die Elementarzelle dargestellt, das ist der kleinstmögliche Block von Atomen,
der dann gestapelt den Kristall ergibt. Sie hat in diesem Fall annähernd die Form eines Quaders.
Dargestellt ist die monokline Modifikation des Ethans bei 85 K. Bei etwa 89,9 K geht diese Modifikation in eine plastische Modifikation
mit kubischem Kristallgitter über, in der die Position der Wasserstoffatome aufgrund der vermehrten Wärmebewegung nicht mehr
festgelegt ist, um dann bei ca. 90,3 K zu schmelzen.
Unklar ist, ob es die in der ältern Literatur (H Mark & E Poland (1925), Z. Kristallogr. 62, 103-112) behauptete hexagonale
Modifikation des Ethans gibt.
Warum haben in der homologen Reihe der Alkane diejenigen mit ungeradzahliger Anzahl von C-Atomen einen niedrigeren Schmelzpunkt? Es
liegt an der Stapelung der Moleküle. Hier liegen Ethanmoleküle jeweils parallel in Schichten, die gegeneinander um
90o verdreht sind.
Für die Details siehe folgende Publikation:
Roland Boese, Hans-Christoph Weiss, Dieter Bläser (1999): The Melting Point Alternation in the Short-Chain n-Alkanes:
Single-Crystal X-Ray Analyses of Propane at 30 K and of n-Butane to n-Nonane at 90 K. Angew. Chem. Int. Ed. 38(7), 988-992.
Die Stapelung von Ethan ist so beschaffen, dass die Moleküle 58,9% der Elementarzelle füllen (bei 85 K).
Betrachten Sie die Kristallstruktur auch bei der Darstellung mit 100% Raumfüllung der Atome! Vergleichen Sie außerdem
mit den anderen Alkanen.
Die Darstellung beruht auf eigenen Berechnungen nach den kristallographischen Daten in: GJH van Nes & A Vos (1978): Single-Crystal
Structures and Electron Density Distributions of Ethane, Ethylene and Acetylene. I. Single-Crystal X-ray Structure Determinations of
Two Modifications of Ethane. Acta Crystallogr, Sect. B, vol 34, pp. 1947ff.
Propan als Feststoff
Kohlenstoff ist grau dargestellt, Wasserstoff weiß.
Formel: C3H8
Es wird auch die Elementarzelle dargestellt, das ist der kleinstmögliche Block von Atomen,
der dann gestapelt den Kristall ergibt. Sie hat in diesem Fall annähernd die Form eines Quaders.
Warum haben in der homologen Reihe der Alkane diejenigen mit ungeradzahliger Anzahl von C-Atomen einen niedrigeren
Schmelzpunkt? Es liegt an der Stapelung der Moleküle. Die Propanmoleküle liegen in leicht welligen Schichten,
die parallel zur b-Achse und zur Diagonale zwischen der a- und c-Achse liegen. Innerhalb der Schichten (Blick parallel a)
stapeln sie sich verdreht und verschachtelt.
Für die Details siehe folgende Publikation:
Roland Boese, Hans-Christoph Weiss, Dieter Bläser (1999): The Melting Point Alternation in the Short-Chain n-Alkanes:
Single-Crystal X-Ray Analyses of Propane at 30 K and of n-Butane to n-Nonane at 90 K. Angew. Chem. Int. Ed. 38(7), 988-992.
Auf den kristallographischen Daten dieser Publikation beruhen auch die Berechnungen für diese Darstellung.
Die Stapelung von Propan ist so beschaffen, dass die Moleküle (extrapoliert für eine Temperatur von 90 K)
nur 58,6% der Elementarzelle füllen. Folglich sind die Abstände zwischen den
Molekülen insgesamt größer als z.B. bei Butan, und die Van-der-Waals-Kräfte
entsprechend schwächer.
Betrachten Sie die Kristallstruktur auch bei der Darstellung mit 100% Raumfüllung der Atome! Vergleichen Sie
außerdem mit den anderen Alkanen.
Butan als Feststoff
Kohlenstoff ist grau dargestellt, Wasserstoff weiß.
Formel: C4H10
Es wird auch die Elementarzelle dargestellt, das ist der kleinstmögliche Block von Atomen,
der dann gestapelt den Kristall ergibt. Sie hat in diesem Fall annähernd die Form eines Quaders.
Warum haben in der homologen Reihe der Alkane diejenigen mit geradzahliger Anzahl von C-Atomen einen höheren
Schmelzpunkt? Es liegt an der Stapelung der Moleküle. Die Anordnung ähnelt derjenigen im Ethankristall, allerdings
sind die Winkel schiefer.
Für die Details siehe folgende Publikation:
Roland Boese, Hans-Christoph Weiss, Dieter Bläser (1999): The Melting Point Alternation in the Short-Chain n-Alkanes:
Single-Crystal X-Ray Analyses of Propane at 30 K and of n-Butane to n-Nonane at 90 K. Angew. Chem. Int. Ed. 38(7), 988-992.
Auf den kristallographischen Daten dieser Publikation beruhen auch die Berechnungen für diese Darstellung.
Die Stapelung von Butan ist so beschaffen, dass die Moleküle 61,3% der Elementarzelle füllen (bei 90 K). Folglich sind
die Abstände zwischen den Molekülen insgesamt kleiner als z.B. bei Propan, und die Van-der-Waals-Kräfte
entsprechend größer.
Fester, kristalliner Butan kommt in 3 Modifikationen vor: Butan I ist zwischen 108 K und 135 K stabil. In dieser Modifikation
können die Butanmoleküle frei um ihrer Längsachse rotieren; der Kristall ist also weniger geordnet (plastischer Kristall).
Unterhalb von 105 K existieren die metastabile Phase Butan II (deren Struktur hier gezeigt wird) und die stabile Phase Butan III.
Betrachten Sie die Kristallstruktur auch bei der Darstellung mit 100% Raumfüllung der Atome! Vergleichen Sie außerdem
mit den anderen Alkanen.
Pentan als Feststoff
Kohlenstoff ist grau dargestellt, Wasserstoff weiß.
Formel: C5H12
Es wird auch die Elementarzelle dargestellt, das ist der kleinstmögliche Block von Atomen,
der dann gestapelt den Kristall ergibt. Sie hat in diesem Fall annähernd die Form eines Quaders.
Warum haben in der homologen Reihe der Alkane diejenigen mit ungeradzahliger Anzahl von C-Atomen einen niedrigeren Schmelzpunkt? Es
liegt an der Stapelung der Moleküle. In dieser Kristallstruktur bilden die Pentanmoleküle stufige Schichten, innerhalb
derer sie parallel angeordnet sind. Die einzelnen Schichten liegen schräg übereinander. Der Winkel zwischen den Längsachsen
der Moleküle aus benachbarten Schichten beträgt ca. 37o.
Für die Details siehe folgende Publikation:
Roland Boese, Hans-Christoph Weiss, Dieter Bläser (1999): The Melting Point Alternation in the Short-Chain n-Alkanes: Single-Crystal
X-Ray Analyses of Propane at 30 K and of n-Butane to n-Nonane at 90 K. Angew. Chem. Int. Ed. 38(7), 988-992. Auf den kristallographischen
Daten dieser Publikation beruhen auch die Berechnungen für diese Darstellung.
Die Stapelung von Pentan ist so beschaffen, dass die Moleküle 64,8% der Elementarzelle füllen (bei 90 K).
Betrachten Sie die Kristallstruktur auch bei der Darstellung mit 100% Raumfüllung der Atome! Vergleichen Sie außerdem mit den
anderen Alkanen.
Hexan als Feststoff
Kohlenstoff ist grau dargestellt, Wasserstoff weiß.
Formel: C6H14
Es wird auch die Elementarzelle dargestellt, das ist der kleinstmögliche Block von Atomen,
der dann gestapelt den Kristall ergibt. Sie hat in diesem Fall annähernd die Form eines Quaders.
Warum haben in der homologen Reihe der Alkane diejenigen mit geradzahliger Anzahl von C-Atomen einen höheren Schmelzpunkt? Es liegt
an der Stapelung der Moleküle. Für die Details siehe folgende Publikation:
Roland Boese, Hans-Christoph Weiss, Dieter Bläser (1999): The Melting Point Alternation in the Short-Chain n-Alkanes: Single-Crystal
X-Ray Analyses of Propane at 30 K and of n-Butane to n-Nonane at 90 K. Angew. Chem. Int. Ed. 38(7), 988-992. Auf den
kristallographischen Daten dieser Publikation beruhen auch die Berechnungen für diese Darstellung.
Die Stapelung von Hexan ist so beschaffen, dass alle Moleküle parallel zueinander liegen. Man kann auch hier wieder Schichten erkennen.
Im Grundriss einer Schicht hat das Hexanmolekül eine etwa parallelogrammförmige Gestalt (drehen Sie die Ansicht so, dass Sie
genau von vorne auf die Schicht sehen). Trotz des Versatzes zwischen den Parallelogrammen — der durch den größeren
Abstand zwischen den endständigen Methylgruppen entsteht — ist die Stapelung besser als bei ungeradzahligen Alkanen dieses
Kristalltyps, weshalb die Van-der-Waals-Kräfte vergleichsweise stärker sind. Die Hexanmoleküle füllen
65,6% der Elementarzelle (bei 90 K). Vergleichen Sie hierzu auch die Struktur von Heptan.
Beginnend mit Hexan kristallisieren die Alkane mit gerader Anzahl von C-Atomen alle nach dem gleichen Schema, wie auch
beginnend mit Heptan die Alkane mit ungerader Anzahl von C-Atomen nach dem jeweils gleichen Schema, aber
abweichend vom geradzahligen Schema, kristallisieren. Man
kann also sagen, dass das Zick-Zack in der Schmelzkurve der Alkane erst ab Hexan einen systematischen
Grund hat; bei den kürzeren Alkanen ergibt es sich zufällig.
Octan krisallisiert nach dem gleichen Muster wie Hexan.
Betrachten Sie die Kristallstruktur auch bei der Darstellung mit 100% Raumfüllung der Atome! Vergleichen Sie außerdem
mit den anderen Alkanen.
Heptan als Feststoff
Kohlenstoff ist grau dargestellt, Wasserstoff weiß.
Formel: C7H16
Es wird auch die Elementarzelle dargestellt, das ist der kleinstmögliche Block von Atomen,
der dann gestapelt den Kristall ergibt. Sie hat in diesem Fall annähernd die Form eines Quaders.
Warum haben in der homologen Reihe der Alkane diejenigen mit ungeradzahliger Anzahl von C-Atomen einen niedrigeren Schmelzpunkt? Es liegt
an der Stapelung der Moleküle. Für die Details siehe folgende Publikation:
Roland Boese, Hans-Christoph Weiss, Dieter Bläser (1999): The Melting Point Alternation in the Short-Chain n-Alkanes: Single-Crystal
X-Ray Analyses of Propane at 30 K and of n-Butane to n-Nonane at 90 K. Angew. Chem. Int. Ed. 38(7), 988-992. Auf den kristallographischen
Daten dieser Publikation beruhen auch die Berechnungen für diese Darstellung.
Die Stapelung vonn Heptan ist so beschaffen, dass alle Moleküle parallel zueinander liegen. Man kann auch hier wieder Schichten
erkennen. Im Grundriss der Schicht hat das Heptanmolekül eine etwa trapezförmige Gestalt (drehen Sie die Ansicht so, dass Sie
genau von vorne auf die Schicht sehen). Diese Trapeze sind nun so angeordnet, dass zwischen den endständigen Methylgruppen ein
relativ großer Abstand besteht. Dadurch kommt es zu einem Versatz zwischen den Trapezen und zu einer insgesamt lockeren Stapelung,
weshalb die Van-der-Waals-Kräfte vergleichsweise schwach sind. Die Heptanmoleküle füllen
65,3% der Elementarzelle (bei 90 K). Vergleichen Sie hierzu auch die Struktur von Hexan.
Nonan krisallisiert nach dem gleichen Muster wie Heptan.
Betrachten Sie die Kristallstruktur auch bei der Darstellung mit 100% Raumfüllung der Atome! Vergleichen
Sie außerdem mit den anderen Alkanen.
Cyclopentan als Feststoff
Kohlenstoff ist grau dargestellt, Wasserstoff weiß.
Formel: C5H10
Es wird auch die Elementarzelle dargestellt, das ist der kleinstmögliche Block von Atomen,
der dann gestapelt den Kristall ergibt. Sie hat in diesem Fall die Form eines Parallelepipeds.
Es ist schwieriger, ringförmige Moleküle zu stapeln, als linerare Moleküe. Es ist klar,
dass das zu einer lockeren Stapelung führt .
Die Darstellung beruht auf eigenen Berechnungen nach den kristallographischen Daten in:
Torrisi, Antonio et al. (2008): Solid phases of cyclopentane: combined experimental and simulation study.
The journal of physical chemistry B, 112 (12), 3746-3758.
Betrachten Sie die Kristallstruktur auch bei der Darstellung mit 100% Raumfüllung der Atome! Vergleichen Sie außerdem
mit den linearen Alkanen.
Gips (Calciumsulfat Dihydrat)
Die Calciumionen sind grün dargestellt, Schwefel gelb, Sauerstoff rot, Wasserstoff weiß.
Formel: CaSO4 · 2 H2O
Die Dipol-Ion-Wechselwirkungen zwischen Calciumionen und
Sauerstoff sind in Cyan dargestellt.
Es wird auch die Elementarzelle dargestellt, das ist der kleinstmögliche Block von Atomen,
der dann gestapelt den Kristall ergibt. Sie hat in diesem Fall die Form eines Parallelepipeds (verzogener Quader).
Dieses Kristallgitter dient als Beispiel für eine Substanz, die Kristallwasser enthält.
Vergleichen Sie auch die Struktur des wasserfreien Calciumsulfats (Mineralname: Anhydrit).
Die Darstellung beruht auf eigenen Berechnungen nach den kristallographischen Daten in: Comodi P, Nazzareni S, Zanazzi PF, Speziale S (2008):
High-pressure behavior of gypsum: A single-crystal X-ray study. American Mineralogist 93, 1530-1537.
Anhydrit (Calciumsulfat)
Die Calciumionen sind grün dargestellt, Schwefel gelb, Sauerstoff rot.
Formel: CaSO4
Es wird auch die Elementarzelle dargestellt, das ist der kleinstmögliche Block von Atomen,
der dann gestapelt den Kristall ergibt. Sie hat in diesem Fall die Form eines Quaders.
Vergleichen Sie auch die Struktur des wasserhaltigen Calciumsulfat-Dihydrats (Mineralname: Gips).
Die Darstellung beruht auf eigenen Berechnungen nach den kristallographischen Daten in: Hawthorne FC, Ferguson RB (1975):
Anhydrous sulphates. II. Refinement of the crystal structure of anhydrite. The Canadian Mineralogist 13, 289-292.
Wasserfreies Kupfersulfat
Kupfer ist bräunlich dargestellt, Schwefel gelb, Sauerstoff rot.
Formel: CuSO4
Die Ion-Ion-Wechselwirkungen zwischen Kupferionen und
Sauerstoff sind in Cyan dargestellt.
Es wird auch die Elementarzelle dargestellt, das ist der kleinstmögliche Block von Atomen,
der dann gestapelt den Kristall ergibt. Sie hat in diesem Fall die Form eines Quaders.
Vergleichen Sie auch die Struktur des wasserhaltigen Kupfersulfat-Pentahydrats.
Die Darstellung beruht auf eigenen Berechnungen nach den kristallographischen Daten in: Wildner M, Giester G (1988): Crystal structure
refinements of synthetic chalcocyanite (CuSO4) and zincosite (ZnSO4). Mineralogy and Petrology 39 201-209.
Kupfersulfat Pentahydrat
Kupfer ist bräunlich dargestellt, Schwefel gelb, Sauerstoff rot, Wasserstoff weiß.
Formel: CuSO4 · 2 H2O
Die Wasserstoffbrückenbindungen und die Dipol-Ion-Wechselwirkungen zwischen Kupferionen und
Sauerstoff sind in Cyan dargestellt.
Es wird auch die Elementarzelle dargestellt, das ist der kleinstmögliche Block von Atomen,
der dann gestapelt den Kristall ergibt. Sie hat in diesem Fall die Form eines Parallelepipeds (verzogener Quader).
Dieses Kristallgitter dient als Beispiel für eine Substanz, die Kristallwasser enthält.
Vergleichen Sie auch die Struktur des wasserfreien Kupfersulfats.
Die Darstellung beruht auf eigenen Berechnungen nach den kristallographischen Daten in: Bacon GE, Titterton DH (1975):
Neutron-diffraction studies of CuSO4*5(H2O) and CuSO4*5(D2O). Zeitschrift fur Kristallographie 141, 330-341.
Eis Ih
Sauerstoff ist rot dargestellt, Wasserstoff weiß.
Formel: H2O
Die Wasserstoffbrückenbindungen sind gelb gestrichelt dargestellt.
Dies ist das Eis, das wir alle kennen. Je nach den Druck- und Temperaturbedingungen gibt es nämlich verschiedene Arten, wie Wasser
kristallisiert.
Wie die Druck- und Temperaturbereiche, in denen die verschiedenen Modifikationen des Eises stabil sind, voneinander abzugrenzen sind,
kann man dem Phasendiagramm des Wassers entnehmen.
In vielen der Modifikationen des Eises, so auch im Eis Ih, sind die Positionen der Wasserstoffatome nicht festgelegt. Wie man es auch
von flüssigem Wasser kennt, können die Wassermoleküle nämlich Protonen austauschen. Für jedes Sauerstoffatom
gibt es im Eis Ih vier Positionen im Kristallgitter, auf denen Wasserstoffatome sitzen können. In der nebenstehenden Darstellung
sind alle 4 Positionen zu erkennen. Die Verteilung der Wasserstoffatome auf die Positionen erfolgt zufällig. In dieser Darstellung
wurden dementsprechend die Positionen willkürlich ausgewählt. Nicht besetzte Positionen sind transparent dargestellt.
Die Struktur von Eis Ih ist sehr locker; große sechseckige Kanäle durchziehen den Kristall in alle Richtungen. Der Zusammenhalt
wird durch Wasserstoffbrückenbindungen gewährleistet. Diese lockere Struktur bedingt die geringe Dichte des Eises (Eis schwimmt auf Wasser).>
Eis II
Sauerstoff ist rot dargestellt, Wasserstoff weiß.
Formel: H2O
Die Wasserstoffbrückenbindungen sind gelb gestrichelt dargestellt.
Eis II ist eine weitere Modifikation des Eises. Je nach den Druck- und Temperaturbedingungen gibt es nämlich verschiedene Arten,
wie Wasser kristallisiert. Eis II entsteht bei höheren Drücken als das "normale" Eis Ih.
Wie die Druck- und Temperaturbereiche, in denen die verschiedenen Modifikationen des Eises stabil sind, voneinander abzugrenzen sind,
kann man dem Phasendiagramm des Wassers entnehmen.
Eis VII
Sauerstoff ist rot dargestellt, Wasserstoff weiß.
Formel: H2O
Die Wasserstoffbrückenbindungen sind gelb gestrichelt dargestellt.
Eis VII ist eine weitere Modifikation des Eises. Je nach den Druck- und Temperaturbedingungen gibt es nämlich verschiedene Arten,
wie Wasser kristallisiert. Eis VII entsteht bei noch höheren Drücken als Eis II und ist, wegen des hohen Druckes, auch bei
Temperaturen über 0o C stabil.
Wie die Druck- und Temperaturbereiche, in denen die verschiedenen Modifikationen des Eises stabil sind, voneinander abzugrenzen sind,
kann man dem Phasendiagramm des Wassers
entnehmen.
Unter so hohem Druck sind lockere Strukturen wie Eis Ih und Eis II energetisch nicht günstig. Eis VII ist deshalb auch viel dichter
gepackt und hat eine viel höhere Dichte.
In vielen der Modifikationen des Eises, so auch im Eis VII, sind die Positionen der Wasserstoffatome nicht festgelegt. Wie man es auch von
flüssigem Waser kennt, können die Wassermoleküle nämlich Protonen austauschen. Für jedes Sauerstoffatom
gibt es im Eis VII vier Positionen im Kristallgitter, auf denen Wasserstoffatome sitzen können. In der nebenstehenden
Darstellung sind alle 4 Positionen zu erkennen. Die Verteilung der Wasserstoffatome auf die Positionen erfolgt zufällig.
In dieser Darstellung wurden dementsprechend die Positionen willkürlich ausgewählt. Nicht besetzte Positionen sind
transparent dargestellt.
Cellulose Iβ
Kohlenstoff ist grau dargestellt, Wasserstoff weiß, Sauerstoff rot.
Wasserstoffbrückenbindungen sind gelb gestrichelt.
Es wird auch die Elementarzelle dargestellt, das ist der kleinstmögliche Block von Atomen,
der dann gestapelt den Kristall ergibt. Sie hat in diesem Fall annähernd die Form eines Quaders.
Cellulose ist der Hauptbestandteil pflanzlicher Zellwände und deshalb auch der Hauptbestandteil
pflanzlicher Fasern, z.B. Baumwolle.
Das Polysaccharid Cellulose besteht aus D-Glucoseeinheiten, die über
1,4-β-glycosidische Bindungen verknüpft sind.
Natürliche Cellulose kommt in den beiden Kristallstrukturen Cellulose Iα und
Cellulose Iβ vor, die sich geringfügig in der Stapelung der Schichten unterscheiden. Innerhalb der Schichten wird
die Struktur durch Wasserstoffbrückenbindungen zusammengehalten; zwischen den Schichten wirken lediglich
Van-der-Waals-Kräfte.
Die Polysaccharidketten verlaufen parallel zur c-Achse der Elementarzelle; es werden jeweils 3 bzw. 2 Monosaccharidbausteine
dargestellt.
Cellulose in pflanzlichen Zellwänden oder in Pflanzenfasern (z.B. Baumwolle) besteht aus kristallinen Bereichen und aus
Bereichen, in denen die Polysaccharidketten ungeordnet sind.
Die Darstellung beruht auf eigenen Berechnungen nach den kristallographischen Daten in:
Yoshiharu Nishiyama, Paul Langan and Henri Chanzy,
Crystal Structure and Hydrogen-Bonding System in Cellulose
Iβ from Synchrotron X-ray and Neutron Fiber Diffraction.
J. Am. Chem. Soc. 2002 (124), S. 9074-9082.
Cellulose II
Kohlenstoff ist grau dargestellt, Wasserstoff weiß, Sauerstoff rot.
Wasserstoffbrückenbindungen sind gelb gestrichelt.
Es wird auch die Elementarzelle dargestellt, das ist der kleinstmögliche Block von Atomen,
der dann gestapelt den Kristall ergibt. Sie hat in diesem Fall die Form eines Parallelepipeds.
Gezeigt wird die kristalline Form der mercerisierten Cellulose. Cellulose besteht aus D-Glucoseeinheiten, die über
1,4-β-glycosidische Bindungen verknüpft sind. Bei der Mercerisierung werden Cellulosefasern (Baumwolle) unter
Spannung mit Natronlauge behandelt.
Die Polysaccharidketten verlaufen parallel zur c-Achse der Elementarzelle. Von Lage zu Lage wechselt ihre Richtung
(antiparallele Anordnung).
Wasserstoffbrückenbindungen gibt es sowohl innerhalb der Polysaccharidketten –
dadurch werden die Ketten stablisiert –, als auch zwischen den Ketten innerhalb der Lagen, die sich parallel zur
a-b-Ebene der Elementarzelle erstrecken. Dies ist genau so wie bei der natürlch vorkommenden Cellulose I.
.
Im Gegensatz zur Cellulose I gibt es aber auch zwischen den Lagen Wasserstoffbrückenbindungen.
Darauf beruht die höhere Festigkeit und der Glanz der mercerisierten Baumwolle.
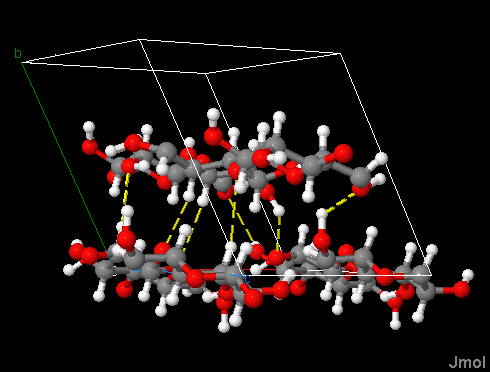
Die Darstellung beruht auf eigenen Berechnungen nach den kristallographischen Daten in:
Paul Langan, Yoshiharu Nishiyama, and Henri Chanzy (2001):
A Revised Structure and Hydrogen-Bonding System in Cellulose II
from a Neutron Fiber Diffraction Analysis.
J. Am. Chem. Soc. 2003 (125), 14300-14306
β-Chitin
Kohlenstoff ist grau dargestellt, Wasserstoff weiß, Sauerstoff rot, Stickstoff blau.
Wasserstoffbrückenbindungen sind gelb gestrichelt.
Es wird auch die Elementarzelle dargestellt, das ist der kleinstmögliche Block von Atomen, der dann
gestapelt den Kristall ergibt. Sie hat in diesem Fall annähernd die Form eines Quaders.
Chitin ist das Material, aus dem die Außenskelette der Insekten und anderer Gliederfüßer
sowie die Zellwand bei Pilzen gemacht sind. Hier wird β-Chitin dargestellt. Es ist die seltenere Modifikation des Chitins und kommt
bei Tintenfischen, Diatomeen, Pogonophoren und Vestimentiferen vor. β-Chitin hat die
Besonderheit, Wassermoleküle in sein Kristallgitter einzulagern und aufzuquellen.
Es handelt sich um ein Derivat des Polysaccharids Cellulose. Chitin besteht aus D-Glucoseeinheiten, bei denen
am 2. Kohlenstoffatom statt einer Hydroxygruppe die Gruppe —NH—CO—CH3 substituiert ist.
Diese Einheiten sind mit 1,4-β-glycosidischen Bindungen verknüpft.
Hier wird β-Chitin dargestellt. Die Polysaccharidketten verlaufen
parallel zur c-Achse der Elementarzelle; es werden jeweils 2 Monosaccharidbausteine
dargestellt.
Die kristallographischen Daten, auf denen die Berechnungen für diese Darstellung beruhen,
entstammen der Cambridge Structural Database (http://www.ccdc.cam.ac.uk; Archivnr. 1425611).
α-Chitin
Kohlenstoff ist grau dargestellt, Sauerstoff rot, Stickstoff blau; Wasserstoff fehlt in diesen Daten;
Wasserstoffbrückenbindungen sind gelb gestrichelt.
Es wird auch die Elementarzelle dargestellt, das ist der kleinstmögliche Block von Atomen,
der dann gestapelt den Kristall ergibt. Sie hat in diesem Fall die Form eines Quaders.
Chitin ist das Material, aus dem die Außenskelette der Insekten und anderer Gliederfüßer
sowie die Zellwand bei Pilzen gemacht sind.
Es handelt sich um ein Derivat des Polysaccharids Cellulose. Chitin besteht aus D-Glucoseeinheiten,
bei denen am 2. Kohlenstoffatom statt einer Hydroxygruppe die Gruppe
—NH—CO—CH3 substituiert ist.
Diese Einheiten sind mit 1,4-β-glycosidischen Bindungen verknüpft.
Hier wird α-Chitin dargestellt, die häufigere Modifikation des Chitins. Die
Polysaccharidketten verlaufen parallel zur c-Achse der Elementarzelle; es werden jeweils 2
Monosaccharidbausteine dargestellt.
Im Kristallgitter gibt es für einige der Sauerstoffatome zwei mögliche
Plätze. Die Verteilung der Sauerstoffatome auf diese Positionen erfolgt zufällig.
In dieser Darstellung wurden diese Positionen transparent dargestellt.
Die kristallographischen Daten, auf denen die Berechnungen für diese Darstellung beruhen,
entstammen dem Aufsatz von Ram Minke & John Blackwell (1978), The Structure of α-Chitin,
J. Mol. Biol. 120, 167-181. Damals war es noch schwierig, die Positionen der Wasserstoffatome
zu bestimmen, die deshalb fehlen. Wasserstoffbrückenbindungen sind folglich zwischen je
zwei Sauerstoffatomen bzw. zwischen Stickstoff- und Sauerstoffatomen dargestellt.
This site is about the following topics:
- • the crystal structure of solid alkanes and it's relationship to the melting point curve;
- • how are water molecules incorporated in a lattice as crystal water;
- • the crystal structure of cellulose, mercerized cellulose and chitin;
- • the structure of ice.
For a better understanding of the spatial relationships, the images can be turned in space using the mouse.
The image can be enlarged using the mouse wheel.
On the bottom of the page you can select different substances and change the way atoms are rendered.
Please use the menus below.
Solid methane
Carbon is displayed in grey, hydrogen in white.
Formula: CH4
The unit cell is on display as well. It is the smallest possible block of atoms
building up the crystal. In the present case it's shape is cubic.
On display is the modification methan I. This is the modification formed when methane is cooled down at normal athmospheric pressure.
(In the phase diagram shown here, the scale for pressure starts at .01 GPa; this is a 100 times of normal athmospheric pressure!)
Phase diagram of methane. After: R. Bini & G. Pratesi (1997): High-pressure infrared study of solid methane: Phase
diagram up to 30 GPa. Physical Review B, 55 (22), 14800-14809.
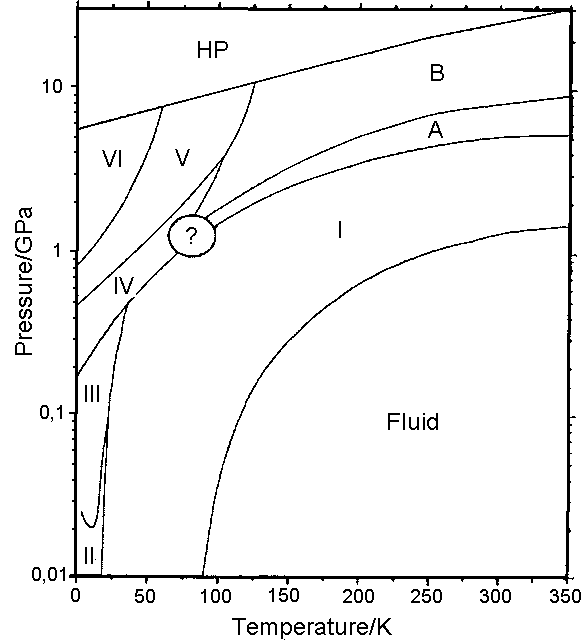
In this modification the methane molecules are able to rotate freely; the positions of the hydrogen atoms thus are not fixed in
the crystal lattice. To represent this, an arbitrary position has been chosen here for each single methane molecule.
Due to this mobility crystals of methane I are plastic.
Upon further cooling the molecules loose their freedom of rotation, resulting in the other modifications.
View the crystal structure also with 100% space filling of the atoms! Besides, compare to the other alkanes.
The visualization is based on my own calculations, using crystallographic data from: A. I. Kitajgorodskij (1955): Organicheskaja
Kristallokhimija. Izdatel'stvo Akademii Nauk SSSR, cited after: Crystallography Open Database (COD), http://www.crystallography.net/
Ethane as a solid
Carbon is displayed in grey, hydrogen in white.
Formula: C2H6
The unit cell is on display as well. It is the smallest possible block of atoms
building up the crystal. In the present case it's shape is rectangular.
On display is the monoclinic modification of ethane at 85 K. At about 89.9 K this modification gives way to a plastic modification
with a cubic lattice, with positions of hydrogen atoms not beeing fixed anymore due to enhanced thermal movement
Again, at 90.3 K this solid will melt.
It remains unclear whether a hexagonal modification of ethane, mentioned in the older literature (H Mark & E Poland (1925), Z. Kristallogr.
62, 103-112), exists.
In the homologous series of alkanes, those with an even number of C atoms are having a slightly higher melting point as compared
to their neighbours. Why is that so? This is due to the stacking of the molecules. In the present case ethane molecules are
lying in parallel layers, rotated around 90o against each other.
For details see the following Paper:
Roland Boese, Hans-Christoph Weiss, Dieter Bläser (1999): The Melting Point Alternation in the Short-Chain n-Alkanes:
Single-Crystal X-Ray Analyses of Propane at 30 K and of n-Butane to n-Nonane at 90 K. Angew. Chem. Int. Ed. 38(7), 988-992.
Ethane molecules are stacked in a way resulting in about 58.9 % space fill (at a temperature of 85 K).
View the crystal structure also with 100% space filling of the atoms! Besides, compare to the other alkanes.
The visualization is based on my own calculations, using crystallographic data in: GJH van Nes & A Vos (1978): Single-Crystal
Structures and Electron Density Distributions of Ethane, Ethylene and Acetylene. I. Single-Crystal X-ray Structure
Determinations of Two Modifications of Ethane. Acta Crystallogr, Sect. B, vol 34, pp. 1947ff.
propane as a solid
Carbon is displayed in grey, hydrogen in white.
Formula: C3H8
The unit cell is on display as well. It is the smallest possible block of atoms
building up the crystal. In the present case it's shape is approximately rectangular.
In the homologous series of alkanes, those with an even number of C atoms are having a slightly higher melting point as compared
to their neighbours. Why is that so? This is due to the stacking of the molecules. The propane molecules are lying in slightly
undulating layers, parallel to the b-axis and to the diagonal between a- and c-axis. Within layers (view parallel a)
they are stacked in a somewhat twisted way.
For details see the following Paper:
Roland Boese, Hans-Christoph Weiss, Dieter Bläser (1999): The Melting Point Alternation in the Short-Chain n-Alkanes:
Single-Crystal X-Ray Analyses of Propane at 30 K and of n-Butane to n-Nonane at 90 K. Angew. Chem. Int. Ed. 38(7), 988-992.
On the crystallographic data in this paper and on my own calculations the present visualization is based.
Propane molecules are stacked in a way resulting in about 58.7 % space fill (extrapolated from 30 K to a temperature of 90 K).
Thus overall distances between molecules are
larger than in e.g. butane, and correspondingly the Van-der-Waals forces are weaker.
View the crystal structure also with 100% space filling of the atoms! Besides, compare to the other alkanes.
Butane as a solid
Carbon is displayed in grey, hydrogen in white.
Formula: C4H10
The unit cell is on display as well. It is the smallest possible block of atoms
building up the crystal. In the present case it's shape is approxiatately rectangular.
In the homologous series of alkanes, those with an even number of C atoms are having a slightly higher melting point as compared
to their neighbours. Why is that so? This is due to the stacking of the molecules. The spacial ordering is similar to
ethane, but the angles of the unit cells are more oblique.
For details see the following publication:
Roland Boese, Hans-Christoph Weiss, Dieter Bläser (1999): The Melting Point Alternation in the Short-Chain n-Alkanes:
Single-Crystal X-Ray Analyses of Propane at 30 K and of n-Butane to n-Nonane at 90 K. Angew. Chem. Int. Ed. 38(7), 988-992.
The calculations for the present visualization are based on the crystallographic data in this paper.
The way butane molecules are stacked results in a space filling of 61.3% (at 90 K). Thus distances between molecules
are overall smaller than in e.g. propane, and correspondingly Van-der-Waals forces are larger.
Solid, crystalline butane comes in 3 modifications: butane I, stable between 108 K and 135 K. In this modification
butane molecules can rotate freely around their long axes; the crystal is less ordered (plastic crystal).
Below 105 K there is the metastable phase butane II (the structure shown here) and the stable phase butane III.
Pentane as a solid
Carbon is displayed in grey, hydrogen in white.
Formula: C5H12
The unit cell is on display as well. It is the smallest possible block of atoms
building up the crystal. In the present case it's shape is approximately rectangular.
In the homologous series of alkanes, those with an even number of C atoms are having a slightly higher melting point as compared
to their neighbours. Why is that so? This is due to the stacking of the molecules. In this crystal structure the pentane
molecules are forming kinked layers, lying parallel within layers. These latter are rotated by ca. 37o.
For details see the following Paper:
Roland Boese, Hans-Christoph Weiss, Dieter Bläser (1999): The Melting Point Alternation in the Short-Chain n-Alkanes:
Single-Crystal X-Ray Analyses of Propane at 30 K and of n-Butane to n-Nonane at 90 K. Angew. Chem. Int. Ed. 38(7), 988-992.
On the crystallographic data in this paper and on my own calculations the present visualization is based.
Pentane molecules are stacked in a way resulting in about 64.8% space fill (at a temperature of 90 K).
View the crystal structure also with 100% space filling of the atoms! Besides, compare to the other alkanes.
Hexane as a solid
Carbon is displayed in grey, hydrogen in white.
Formula: C6H14
The unit cell is on display as well. It is the smallest possible block of atoms
building up the crystal. In the present case it's shape is approximately rectangular.
In the homologous series of alkanes, those with an even number of C atoms are having a slightly higher melting point as compared
to their neighbours. Why is that so? This is due to the stacking of the molecules. For details see the following Paper:
Roland Boese, Hans-Christoph Weiss, Dieter Bläser (1999): The Melting Point Alternation in the Short-Chain n-Alkanes:
Single-Crystal X-Ray Analyses of Propane at 30 K and of n-Butane to n-Nonane at 90 K. Angew. Chem. Int. Ed. 38(7), 988-992.
On the crystallographic data in this paper and on my own calculations the present visualization is based.
Hexane molecules are stacked with all molecules lying parallel. Here too layers can be discerned. In ground view
the hexane molecule has the shape of a parallelogram (rotate the view in order to look right on the layers).
In spite of a step between the parallelograms — caused by the larger distance between the terminal methyle
groups — the packing is better than in the odd alkanes of this crystal structure type,
resulting in somewhat stronger Van-der-Waals forces. The hexane molecules fill about
65.6% of the unit cell (at a temperature of 90 K). Compare to the structure of heptane.
Beginning with hexane, all alkanes with an even number of C-Atoms are crystallizing according to the
pattern just described. Also, beginning with heptane, all the alkanes with an odd number of C-Atoms are
crystallizing with an identical pattern, different from that of the even alkanes.
Thus it can be said that the zig-zag of the alkanes' melting curve has a systematic cause only
from a chain lenght of 6 onwards; with the shorter alkanes it happens by chance.
Octane crystalizes in the same pattern as hexane.
View the crystal structure also with 100% space filling of the atoms! Besides, compare to the other alkanes.
Heptane as a solid
Carbon is displayed in grey, hydrogen in white.
Formula: C7H16
The unit cell is on display as well. It is the smallest possible block of atoms
building up the crystal. In the present case it's shape is approximately rectangular.
In the homologous series of alkanes, those with an even number of C atoms are having a slightly higher melting point as compared
to their neighbours. Why is that so? This is due to the stacking of the molecules. For details see the following Paper:
Roland Boese, Hans-Christoph Weiss, Dieter Bläser (1999): The Melting Point Alternation in the Short-Chain n-Alkanes: Single-Crystal
X-Ray Analyses of Propane at 30 K and of n-Butane to n-Nonane at 90 K. Angew. Chem. Int. Ed. 38(7), 988-992. On the crystallographic
data in this paper and on my own calculations the present visualization is based.
Heptane molecules are stacked with all molecules lying parallel. Here too layers can be discerned. In ground view
the heptane molekule has a trapezoidal shape (rotate the view in order to look right on the layers).
The ordering of these trapezoidal shapes results in a relatively large distance between the terminal methyl groups.
This leads to a misalignment between trapezoids and over all to a relatively loose packing,
again weakening the van-der-Waals forces. The heptane molecules fill about
65.3% of the unit cell (at a temperature of 90 K). Compare to the structure of hexane.
Nonane crystalizes in the same pattern as hexane.
View the crystal structure also with 100% space filling of the atoms! Besides, compare to the other alkanes.
Cyclopentane as a solid
Carbon is displayed in grey, hydrogen in white.
Formula: C5H10
The unit cell is on display as well. It is the smallest possible block of atoms
building up the crystal. In the present case it is an parallelepepede.
Stacking ring shaped molecules is more difficult than stacking linear molecules. It is evident
that this leads to a relatively loose packing.
The visualization presented here is based on my own calculations, using crystallographic data from:
Torrisi, Antonio et al. (2008): Solid phases of cyclopentane: combined experimental and simulation study.
The journal of physical chemistry B, 112 (12), 3746-3758.
View the crystal structure also with 100% space filling of the atoms! Besides, compare to the linear alkanes.
Water-free copper sulfate
Copper is displyed in brown, sulfur in yellow, oxygen in red.
Formula: CuSO4
Ion-Ion forces between copper ions and oxygen are displaed in cyan.
The unit cell is on display as well. It is the smallest possible block of atoms
building up the crystal. In the present case it's shape is rectangular.
Compare also ato the structure of copper sulfate pentahydrate, containing crystal water.
The visualization is based on my own calculations, using crystallographic data in: Wildner M, Giester G (1988): Crystal structure
refinements of synthetic chalcocyanite (CuSO4) and zincosite (ZnSO4). Mineralogy and Petrology 39, 201-209.
Copper sulfate pentahydrate
Copper is displayed in brown, sulfur in yellow, oxygen in red, hydrogen in white.
Formula: CuSO4 · 2 H2O
Hydrogen bonds and dipol-ion forces betwees copper ions and oxygen atoms are shown in cyan.
The unit cell is on display as well. It is the smallest possible block of atoms
building up the crystal. In the present case it has the shape of a parallelepiped.
This crystal lattice is an example for a substance containing crystal water.
Compare also to the structure of water-free copper sulfate.
The visualization is based on my own calculations, using crystallographic data in: Bacon GE, Titterton DH (1975):
Neutron-diffraction studies of CuSO4*5(H2O) and CuSO4*5(D2O).
Zeitschrift fur Kristallographie 141, 330-341.
Gypsum (Calcium sulfate dihydrate)
Calcium ions are displayed in green, sulfur in yellow, oxygen in red.
Formula: CaSO4 · 2 H2O
Dipol-Ion-forces betwees calcium ions and oxygen are shown in cyan.
The unit cell is on display as well. It is the smallest possible block of atoms
building up the crystal. In the present case it has the shape of a parallelepiped.
This crystal lalttice serves as an example for a substance containing crystal water.
Compare also to the structure of water-free calcium sulfate (mineral name: anhydrite).
The visualization is based on my own calculations, using crystallographic data in: Comodi P, Nazzareni S, Zanazzi PF, Speziale S (2008):
High-pressure behavior of gypsum: A single-crystal X-ray study. American Mineralogist 93, 1530-1537.
Anhydrite (Calcium sulfate)
Calcium ions are displayed in green, sulfur in yellow, oxygen in red.
Formula: CaSO4
The unit cell is on display as well. It is the smallest possible block of atoms
building up the crystal. In the present case it's shape is cubic.
Compare also to the structure of calcium sulfate-dihydrate (mineral name: gypsum), which contains crystal water.
The visualization is based on my own calculations, using crystallographic data from: Hawthorne FC, Ferguson RB (1975):
Anhydrous sulphates. II. Refinement of the crystal structure of anhydrite. The Canadian Mineralogist 13, 289-292.
Ice VII
Oxygen is displayed in red, hydrogen in white.
Formula: H2O
Hydrogen bonds are dashed yellow.
Ice VII is another modification of ice. Depending on temperature and pressure there are different ways
in which water crystallizes. Ice VII is formed at even higher pressures than ice II and is, due to the high pressure,
stable also at temperatures above 0o C. The precise boundaries between the areas of stability for the
different modifications of ice can be taken from the phase diagram of water.
Under such a high pressure loose structures like ice Ih and ice II are energetically not advantageous. Therefore Ice VII
is packed much more densely and has a higher specific mass.
In many of the modifications of ice, also in ice VII, the positions of hydrogen atoms are not fixed. As known from liquid water,
water molecules can excange protons. For each oxygen atom there are four positions reserved for hydrogen atoms in the
crystal lattice of ice VII. In the visualization shown here all 4 position can be discerned. The distribution
of hydrogen atoms is stochastically. Positions not occupied are shown transparent.
Ice Ih
Oxygen is displayed in red, hydrogen in white.
Formula: H2O
Hydrogen bonds are dashed yellow.
This is the ice we all know. Depending on temperature and pressure there are different ways
in which water crystallizes. The precise boundaries between the areas of stability for the
different modifications of ice can be taken from the phase diagram of water.
In many of the modifications of ice, also in ice Ih, the positions of hydrogen atoms are not fixed. As known from liquid water,
water molecules can excange protons. For each oxygen atom there are four positions reserved for hydrogen atoms in the
crystal lattice of ice VII. In the visualization shown here all 4 position can be discerned. The distribution
of hydrogen atoms is stochastically. Positions not occupied are shown transparent.
The structure of ice Ih is very loose; large hexagonal void canals are transversing the crystal in all direction. The structure
is stabilized by hyrogen bonds. This loose packing of water molecules is the reason for the low specific mass
of ice (ice is floating on water).
Ice II
Oxygen is displayed in red, hydrogen in white.
Formula: H2O
Hydrogen bonds are dashed yellow.
Ice II is another modification of ice. Depending on temperature and pressure there are different ways
in which water crystallizes. Ice II is formed at higher pressures than "normal" ice Ih. The precise boundaries
between the areas of stability for the different modifications of ice can
be taken from the phase diagram of water.
Cellulose Iβ
Carbon is displayed in grey, hydrogen in white, oxygen in red.
Hydrogen bridges are dashed yellow.
The unit cell is on display as well. It is the smallest possible block of atoms
building up the crystal. In the present case it's shape is approximately rectangular.
Cellulose is the main constituent of the cell walls of plants and therefore also of
plant fibres, e.g. cotton.
The polysaccharide cellulose is built up by D-glucose units, linked by
1,4-β-glycosidic bonds.
Natural cellulose exists in two different crystal structures, cellulose Iα and
cellulose Iβ, differing slightly in the stacking of the layers.
Within layers the structure is stabilized and held together
by hydrogen bridges; between layers only
Van-der-Waals forces are present.
The polysacharide chains are running parallel to the c-axis of the unit cell; 3 resp. 2 monosaccharide units
are displayed.
Cellulose in plant material is made up of crystalline parts and also of parts
where the polysaccharide chains are disordered.
The visualization presented here is based on my own calculations, using crystallographic data from::
Yoshiharu Nishiyama, Paul Langan and Henri Chanzy,
Crystal Structure and Hydrogen-Bonding System in Cellulose
β from Synchrotron X-ray and Neutron Fiber Diffraction.
J. Am. Chem. Soc. 2002 (124), S. 9074-9082.
Cellulose II
Carbon is displayed in grey, hydrogen in white, oxygen in red.
Hydrogen bridges are dashed yellow.
The unit cell is on display as well. It is the smallest possible block of atoms
building up the crystal. In the present case it has the shape of a parallelepiped.
On display here is the crystalline form of mercerized cellulose. Cellulose is built up by D-glucose units, linked by
1,4-β-glycosidic bonds. Mercerisation is the treatment of cellulose fibers (cotton) with
caustic soda while kept under tension.
The polysacharide chains are running parallel to the c-axis of the unit cell. From one layer to the next
their direction changes (antiparallel alignment).
In celullose II there are hydrogen bridges within polysaccharide chains –
stabilizing the chains –, as well as between chains within the layers extending parallel
to the a-b plane of the unit cell. This is just as with natural cellulose I.
.
In contrast to cellulose I however there are also hydrogen bridges between layers.
This is the reason for the enhanced tensile strength and luster of mercerized cotton.
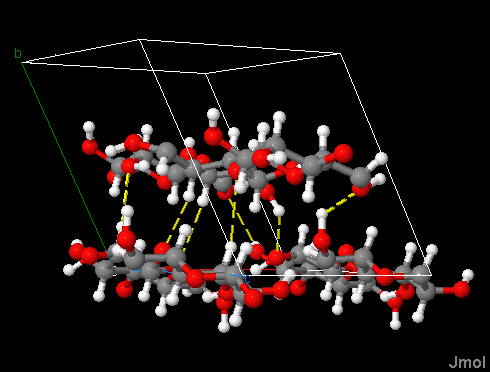
The visualization is based on my own calculations, using crystallographic data from:
Paul Langan, Yoshiharu Nishiyama and Henri Chanzy (2001):
A Revised Structure and Hydrogen-Bonding System in Cellulose II
from a Neutron Fiber Diffraction Analysis.
J. Am. Chem. Soc. 2003 (125), 14300-14306
β-Chitin
Carbon is displayed in grey, hydrogen in white, oxygen in red.
Hydrogen bridges are dashed yellow.
The unit cell is on display as well. It is the smallest possible block of atoms
building up the crystal. In the present case it's shape is approximately rectangular.
Chitin is the material found in the exoskeleton of insects and other arthropoda as well as in the
cell mambrane of fungi. On display here is β-Chitin.
It is the rarer of the two modifications and occurs in squids, some diatomes, pogonophores and
vestimentiferes. A peculiarity of β-chitin is it's ability to take up water molecules.
Chitin is a derivative of the polysaccharide Cellulose. Chitin is built of D-glucose units, having in position 2
the group —NH—CO—CH3 substituted for a hydroxy group.
These units are linked by 1,4-β-glycosidic bonds.
The polysaccharide chains
are running parallel to the c-axis of the unit cell; shown are two monosaccharide units
each.
The visualization is based on my own calculations, using crystallographic data from
the Cambridge Structural Database (http://www.ccdc.cam.ac.uk; archive no. 1425611).
α-Chitin
Carbon is displayed in grey, oxygen in red. Hydrogen is not shown.
Hydrogen bridges are dashed yellow.
The unit cell is on display as well. It is the smallest possible block of atoms
building up the crystal. In the present case it's shape is approximately rectangular.
Chitin is the material found in the exoskeleton of insects and other arthropoda as well as in the
cell mambrane of fungi.
It is a derivative of the polysaccharide Cellulose. Chitin is built of D-glucose units, having in position 2
the group —NH—CO—CH3 substituted for a hydroxi group.
These units are linked by 1,4-β-glycosidic bonds.
On display here is α-cChitin, the modification occuring frequently in nature. The polysaccharide
chains
are running parallel to the c-axis of the unit cell; shown are two monosaccharide units
each.
The crystal lattice has two places for some of the oxygen atoms. The atoms are distributed statistically
among these sites, which are rendered semitransparently.
The visualization is based on my own calculations, using crystallographic data from
Ram Minke & John Blackwell (1978), The Structure of α-Chitin,
J. Mol. Biol. 120, 167-181. Back then it was difficult to determine the positions of the
hydrogen atoms, which therefor are missing. Consequently hydrogen bridges are shown between
the respective oxygen atoms.